16 3.7 Other Environmental Conditions that Affect Growth
Learning Objectives
- Identify and describe different categories of microbes with specific growth requirements other than oxygen, pH, and temperature, such as altered barometric pressure, osmotic pressure, light and nutrient levels
- Give at least one example microorganism for each category of growth requirement
Microorganisms interact with their environment along more dimensions than pH, temperature, and free oxygen levels, although these factors require significant adaptations. We also find microorganisms adapted to varying levels of salinity, barometric pressure, humidity, light and nutrient concentrations.
Osmolarity
All life depends on available water to grow. Available moisture is measured as water activity (aw), which is the ratio of the vapour pressure of the medium of interest to the vapour pressure of pure distilled water; therefore, the aw of water is equal to 1.0. Bacteria require high aw (0.97–0.99), whereas fungi can tolerate drier environments; for example, the range of aw for growth of Aspergillus spp. is 0.8–0.75.
Most natural environments tend to have lower solute concentrations than the cytoplasm of most microorganisms. Rigid cell walls protect the cells from bursting in a dilute environment. Not much protection is available against high osmotic pressure. In this case, water, following its concentration gradient, flows out of the cell. This results in plasmolysis (the shrinking of the protoplasm away from the intact cell wall) and cell death. This fact explains why brines and layering meat and fish in salt are time-honoured methods of preserving food. Microorganisms called halophiles (“salt loving”) actually require high salt concentrations for growth. These organisms are found in marine environments where salt concentrations hover at 3.5%. Extreme halophilic microorganisms, such as the red alga Dunaliella salina and the archaeal species Halobacterium, grow in hypersaline lakes such as the Great Salt Lake (3.5–8 times saltier than the ocean) in Utah (Figure 3.40) and the even saltier Dead Sea (10 times saltier than the ocean).
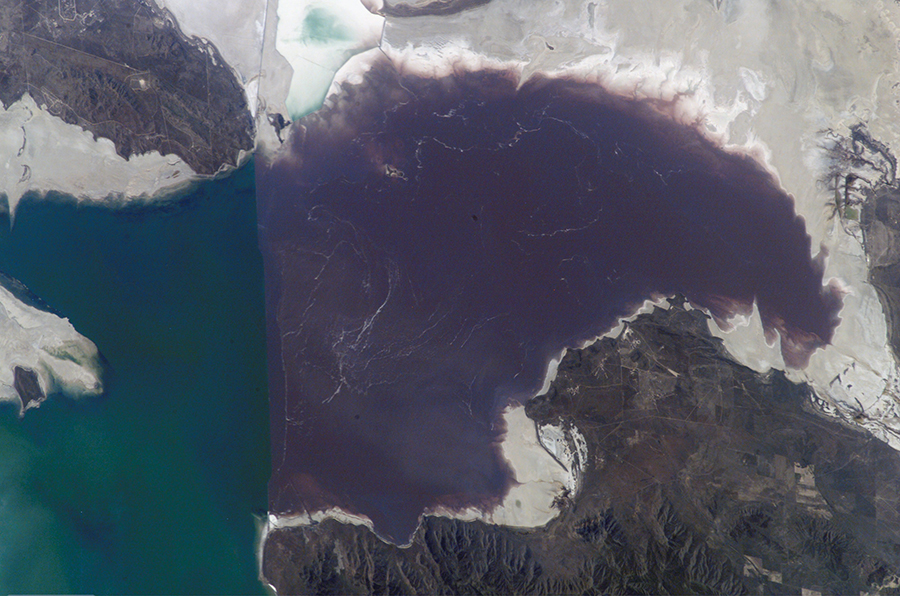
Dunaliella spp. counter the tremendous osmotic pressure of the environment with a high cytoplasmic concentration of glycerol and by actively pumping out salt ions. Halobacterium spp. accumulate large concentrations of K+ and other ions in their cytoplasm. Their proteins are designed for high salt concentrations and lose activity at salt concentrations below 1–2 M. Although most halotolerant organisms, for example Halomonas spp. that grow in salt marshes, do not need high concentrations of salt for growth, they will survive and divide in the presence of high salt. Not surprisingly, the staphylococci, micrococci, and corynebacteria that colonize our skin tolerate salt in their environment. Halotolerant pathogens are an important cause of food-borne illnesses because they survive and multiply in salty food. For example, the halotolerant bacteria S. aureus, Bacillus cereus, and V. cholerae produce dangerous enterotoxins and are major causes of food poisoning.
Osmotic Stress
As described in Section 2.1, cells are subject to changes in osmotic pressure, due to the fact that the plasma membrane is freely permeable to water. In a hypotonic environment, water will pass into the cell, causing the cell to swell and increasing internal pressure. If the situation is not rectified then the cell will eventually burst from lysis of the plasma membrane. Conversely, if the solute concentration of the environment is hypertonic, water will leave the cell, causing the cell to dehydrate. Extended periods of dehydration will cause permanent damage to the plasma membrane (Figure 3.41).

Within limits, microbes can adapt to increases in environmental osmolarity by actively transporting, or synthesizing compatible solutes. These are molecules that can be accumulated to high intracellular concentrations without adversely affecting physiological processes or structures. Examples include K+ and the amino acid proline. Adaptation to a sudden drop in environmental osmolarity may be accomplished by active export of those compatible solutes, as well as through the activation of mechanosensitive channels in the plasma membrane. These channels are thought to open as a result of increased turgor pressure (pressure of the plasma membrane against the rigid cell wall), resulting in the leakage of cytoplasmic solutes and the lowering of intracellular osmolarity. Extreme fluctuations will trigger a global stress response which includes the induction of chaperone proteins. Extremely low water activity environments are, however, lethal to those organisms that spoil food or cause food-borne infections. Decreasing the water content of foods by drying, as in jerky, through freeze-drying or by increasing osmotic pressure, as in brine and jams, are common methods of preventing spoilage.
Pressure
Microorganisms that require high atmospheric pressure for growth are called barophiles. The bacteria and archaea that live at the bottom of the ocean must be able to withstand hydrostatic pressures between 400-1000 atm, in contrast to the 1 atm pressure of sea level. Obtaining and cultivating these organisms is extremely challenging given the depths at which they grow, and the fact most of them have an absolute requirement for high pressure. However the development of various submersibles, sampling and cultivation strategies has gradually helped us begin to characterize these organisms. High pressure has the same effect on the fluidity of cell membranes as does low temperatures, so not surprisingly, barophilic microbes have been found to have increased unsaturated fatty acids in their plasma membrane, as well as shortened fatty acid tails.
Light
Photoautotrophs, such as cyanobacteria or green sulphur bacteria, and photoheterotrophs, such as purple nonsulphur bacteria, depend on sufficient light intensity at the wavelengths absorbed by their pigments to grow and multiply. Energy from light is captured by pigments and converted into chemical energy that drives carbon fixation and other metabolic processes. The portion of the electromagnetic spectrum that is absorbed by these organisms is defined as photosynthetically active radiation (PAR). It lies within the visible light spectrum ranging from 400 to 700 nanometers (nm) and extends in the near infrared for some photosynthetic bacteria. A number of accessory pigments, such as fucoxanthin in brown algae and phycobilins in cyanobacteria, widen the useful range of wavelengths for photosynthesis and compensate for the low light levels available at greater depths of water. Other microorganisms, such as the archaea of the class Halobacteria, use light energy to drive their proton and sodium pumps. The light is absorbed by a pigment protein complex called bacteriorhodopsin, which is similar to the eye pigment rhodopsin. Photosynthetic bacteria are present not only in aquatic environments but also in soil and in symbiosis with fungi in lichens. The peculiar sight of pink snow in Figure 3.42, also called watermelon snow, is caused by a microalga Chlamydomonas nivalis, a green alga rich in a secondary red carotenoid pigment (astaxanthin) which gives the pink hue to the snow where the alga grows.
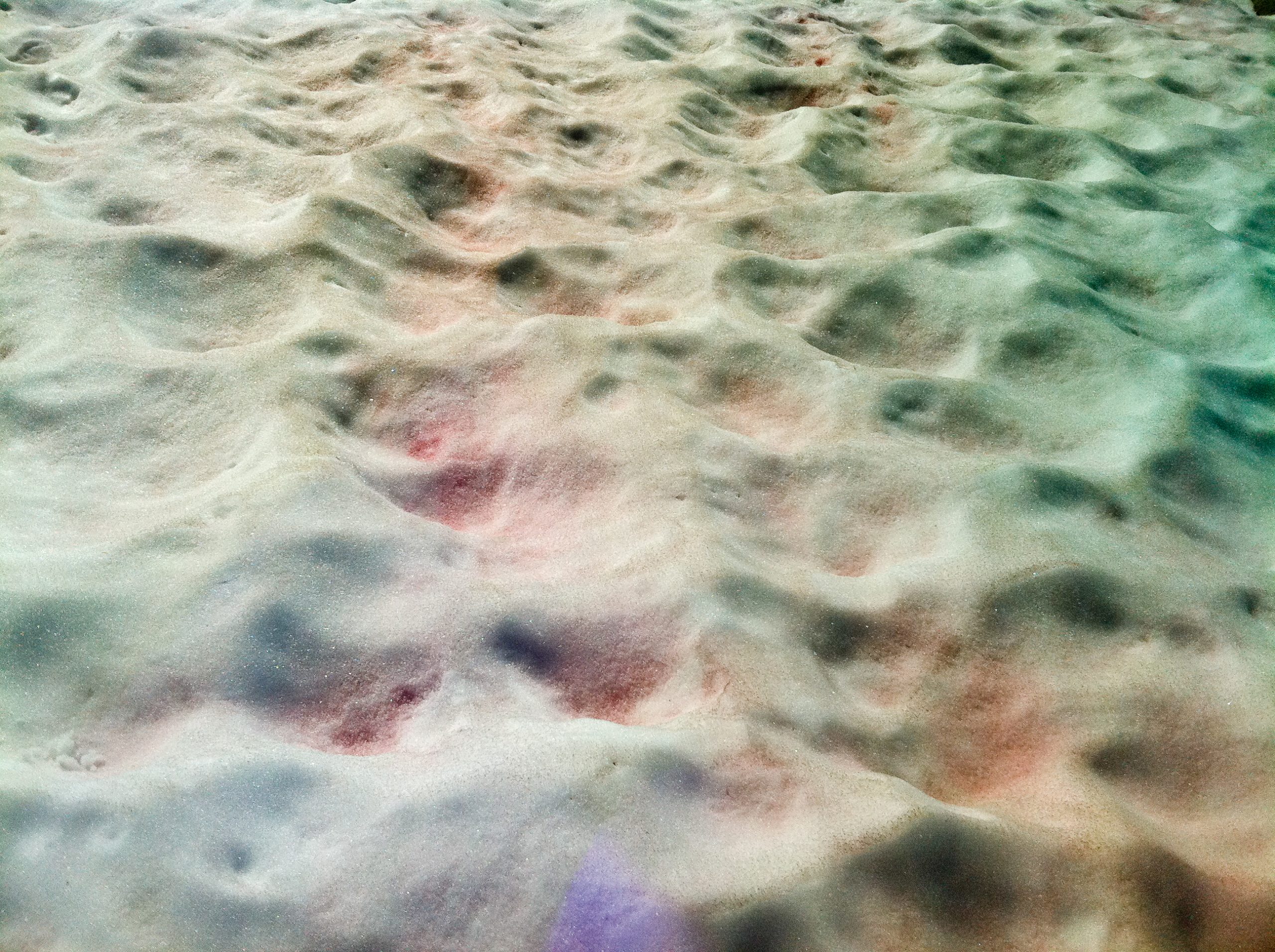
Nutrient Levels
While it is probably self-evident that growth requires access to an adequate supply of energy and carbon sources, as well as other nutrients required to build biomass, it is perhaps counterintuitive that the most prevalent bacteria in the World’s oceans will only grow in the presence of limiting nutrients. Beyond the coastal zones where man’s influence is seen in the higher levels of nutrients, the ocean waters are nutrient-poor: that is, they are oligotrophic. One area that is famously oligotrophic is the Sargasso Sea, located east of the island of Bermuda, in the North Atlantic. This sea is unusual in that is not bounded by any land masses, but is instead defined by four distinct ocean currents (Figure 3.43).
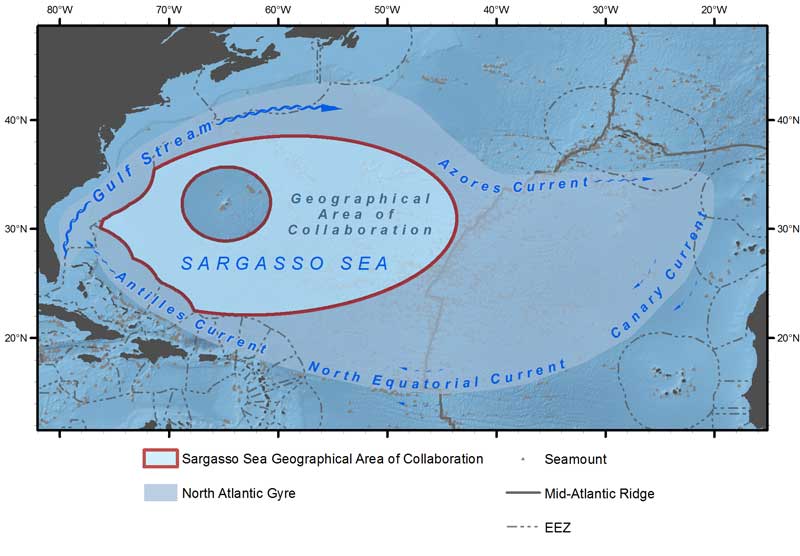
In 2003, J. Craig Venter[1] and colleagues announced the first large-scale shot-gun sequencing project of metagenomic DNA from the Sargasso Sea. As an initial investigation of environmental metagenomic sequencing, they chose this site because of its oligotrophic conditions and an expected resultant low biodiversity. Their results, however, revealed much higher levels of microbial diversity than expected, and identified over a million new genes, including almost 800 new rhodopsin-based photoreceptors. New phylogenetic groups were given the designation SAR, and subsequent metagenomic surveys revealed that most were widespread throughout the World’s oceans. This is particularly true of the SAR11 group of Proteobacteria, which was subsequently shown to be the most abundant heterotrophic bacterium on Earth, with a cosmopolitan distribution in the oceans. Despite this fact, it wasn’t until 2013 that a member of this group, Candidatus Pelagibacter ubique, was cultivated in a defined medium. This bacterium is an extreme oligotroph and was cultivated in an artificial seawater medium[2]. For P. ubique, as well as many marine oligotrophs, despite the fact they may have complex nutrient requirements, high nutrient levels such as those used for the cultivation of most heterotrophs, are actually toxic. P. ubique is one of the marine heterotrophs that express proteorhodopsin. While obligate oligotrophy may be perplexing, the ability to supplement the minimal organic energy sources with solar energy likely provides an explanation for the apparent cosmopolitan distribution of proteorhodopsin genes in the oligotrophic marine environment[3].
- Which photosynthetic pigments were described in this section?
- What is the fundamental stress of a hypersaline environment for a cell?
- What might be an adaptation to growth in oligotrophic environments?
Additional resources
Key Takeaways
- Halophiles require high salt concentration in the medium, whereas halotolerant organisms can grow and multiply in the presence of high salt but do not require it for growth.
- Halotolerant pathogens are an important source of food-borne illnesses because they contaminate foods preserved in salt.
- Photosynthetic bacteria depend on visible light for energy.
- Most bacteria, with few exceptions, require high moisture to grow.
- What are all the descriptive terms used for microbes that live in different environments or the terms used for the environments that they live in? What does each term mean? In what types of environments are each microbial group found?
- What effect does solute concentration have on microbes? How can cells adapt when going from a low solute to a high solute environment and vice versa? What is a compatible solute? What microbial groups have a requirement for high solute concentrations? How do microbes differ in their response to water activity?
- How do microbes differ in their response to pH? What does pH affect in a cell and what do cells that live at high or low pH have to do to survive these conditions?
- How do microbes differ in their response to temperatures? What terms are used for these responses? If a cell is to grow at low or high temperatures, what adaptations does it need to make?
- How do microbes differ in their response to oxygen levels? Why would they differ? What enzymes are needed to adapt to environments containing differing amounts of oxygen?
- How do microbes respond to high pressure? To ionizing radiation? To UV light? What populations are resistant to these conditions?
Multiple Choice
Fill in the Blank
Short Answer
- Fish sauce is a salty condiment produced using fermentation. What type of organism is likely responsible for the fermentation of the fish sauce?
- Venter, J. C., Remington, K., Heidelberg, J.F., Halpern, A.L., Rusch D., Eisen, J.A., Wu, D., Paulsen, I., Nekon, K.E., Netson, W., Fouts, D.E., Levy, S., Knap, A.H., Lomas, M.W., Nealson, K., White, O., Peterson, J., Hoffman, J., Parsons, R., Baden-Tillson, H., Pfannkoch, C., Rogers, Y-H., Smith, H.O. 2004. "Environmental Genome Shotgun Sequencing of the Sargasso Sea." Science 304:66-74 ↵
- Carini, P., Steindler, L., Beszteri, S. and Giovannoni, S.J. 2013. "Nutrient requirements for growth of the extreme oligotroph ‘Candidatus Pelagibacter ubique' HTCC1062 on a defined medium." ISME J. 7:592-602. ↵
- Béjà, O., Spudich, E.N., Spudich, J.L., Leclerc, M., DeLong, E.F. 2001. "Proteorhodopsin phototrophy in the ocean." Nature 411:786-789. ↵